Engineers and building owners often focus on payback periods and return on investment as economic decision-making thresholds for energy efficiency investments. However, there are other parameters that are more accurate and effective for investments in building systems.
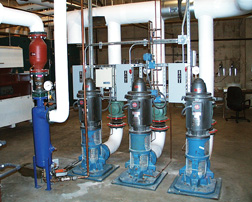
Back in the day, HVAC systems were designed to be stout, longevity was the main focus, and concepts such as operating costs and efficiencies were virtually non-existent. The driving force was purely “How much will it cost and will it last?” Interest rates and return on investments were left to the bankers and stockbrokers.
As the industrial revolution proceeded to where things are today, the world of business began to analyze effective measures to maximize profit. From a construction perspective, there are trade-offs with respect to material and equipment that engage the concepts of first cost and longevity. Out of the necessity to provide a logical method of predicting financial outcomes, the science of economics was born, and the concept of return on investment (ROI).
A famous comedian once remarked that he did not think the science of economics was that complicated. “Buy something and sell it for more.” How hard can that be? Without realizing it, he defined the basic principles of ROI. So if you made a purchase for $100 and sold it for $110 you made $10 or 10%. By today’s analytical standards, that would be simple and to the point. But what if you had to wait two years to get the $10? Is it still 10% or something less because the value of $100 today will change in two years?
Now let’s say you paid $1 a year to store your $100 item; when you sell the item you would want to recover your storage fees, plus the interest value for that time and your profit. This can become a self-perpetuating nightmare of overthinking, overanalyzing, and underpredicting numerous unpredictable variables in an attempt to recover preconceived acceptable return for your investment. If an investment is made on the projection of future gains, the best one can hope for is to break even. This position could even be scrutinized due to the future value as compared to the original investment. This is the nemesis of today’s mechanical engineers.
Building mechanical, electrical, plumbing (MEP), and fire protection systems constitute about 50% of the total facility costs, usually as part of the first cost. Add energy consumption and maintenance costs, and the budget for these engineered systems moves into the range of 60% to 70% of the total cost of ownership. This logically would be an area of strong consideration to hold down expenses, which also creates a somewhat diametrically opposed analysis.
Minimizing the first cost does not always result in lower operating costs. Typically, just the opposite occurs because more durable and efficient equipment has a higher first cost. This is where things get a little woozy.
Generally speaking, codes will define minimum equipment efficiencies. Although the standards are much more stringent than they were 10 years ago, these are defined as minimum standards—in other words, bottom of barrel. Industry organizations such as ASHRAE, U.S. Green Building Council LEED, and U.S. EPA Energy Star provide prescriptive measures in a whole building approach to increase overall building efficiencies while attempting to curtail high investment costs. This inevitability gets to the economic analysis phase. Simple payback is the most common method. Dividing the additional cost of equipment by the first-year savings results in a reasonable snapshot of how long it will take to recover the initial investment. Most businesses will accept a simple payback period of seven years or less; in some areas, three to five years is more common.
Calculating worth
A more sophisticated approach is present worth analysis, which establishes a present time value of all future cash flow by discounting the costs. A typical time frame used for this procedure is 20 years. Oddly enough, this is also the most acceptable industry standard to predict the life of equipment based on ASHRAE data.
Data considered as input includes the future costs of energy, future maintenance costs, replacement costs, life of the product, and future interest rates. There are some pitfalls: prediction of future energy cost is a guessing game, just like predicting stock prices. Two factors, weather and people, are not 100% predictable. Planned maintenance cost can be somewhat consistent, but one catastrophic failure will ruin the budget. In the end, the analysis produces a lifecycle value in today’s dollars. This is then compared to similar investment analyses to select the most reasonable investment. The process can be intense and the projected outcome is always subject to scrutiny.
In some situations, there are incentive programs or rebates that offset the initial first cost based on the projected savings. In many cases, the rebate is given for selecting a higher-efficiency model, covering the difference of the more efficient model, making it a less expensive capital cost. While a good idea to promote efficient design, these programs also can create some rather interesting accounting procedures and create a false value of the enhancement. A more reasonable approach is to let the enhancement stand on its own merit. If there is reasonable ROI, then the rebate or incentive will make the decision more palatable.
In some situations grant money and rebates may be subject to a follow-up review after an operational time period to confirm the savings. If the actual performance does not match the predicted value, that first-cost offset is returned in full or at some prorated value. This becomes problematic because the design team performed an analysis that convinced an owner to invest additional funds assuming there was a first-cost offset. This may become a liability issue for the designer.
Equipment performance
Experience is the best teacher and, over time, design engineers have developed a sixth sense of what works and where real value is generated. This requires an understanding of building dynamics and equipment performance in response to varying loads.
To begin, does the client want to save energy or save operational costs? This might seem a redundant question, but there is a difference. Shifting the operation of equipment to run during off-peak hours does not save energy; it does, however, reduce the operating costs.
A good example is ice storage. These installations have become more prevalent due to the ability to shift cooling production to an off-peak period. The general analysis considers the following:
- A smaller chiller is required because the ice storage offsets a percentage of the occupied hours cooling (reduced first cost)
- Colder chilled water and larger supply/return water differential results in smaller piping, smaller pumps, and smaller coils (reduced first cost)
- Colder supply air temperatures would require smaller ductwork (reduced first cost)
- Ice storage tanks (added first cost)
- Glycol system for low temperature fluid (added first cost)
- Floor space for ice tanks (added first cost)
- Added insulation for piping and ductwork due to low temperatures (added first cost).
The net result of the above is a higher first cost than for a traditional cooling system. An evaluation of the savings needs to be defined. Generally, this type of system will result in slightly higher energy consumption but a lower operating cost. The most obvious savings would be from the absence of a larger chiller operating during the occupied or the utility peak period. Energy and demand charges are significantly reduced. This lower daytime consumption is offset by operating a cooling plant to build ice at night. Pumps, cooling tower fans, and chillers are operating at a higher kW/ton—all to build ice and operate during the unoccupied or off-peak period. The benefit is purely the cost savings due to minimal or no demand charges and reduced energy costs. The ROI for this particular investment is in the five- to seven-year range and specific to the user. There are some things to consider: the success relies heavily on matching the building schedule to the utilities on-/off-peak schedule. It also relies on a level of stability or commitment from the utility regarding the rates and schedules. Selecting an individual piece of equipment on the single merit of its own efficiencies can create significant problems.
A traditional example
New gas boiler efficiencies can exceed 95%. This is common in condensing type units and at low supply water temperatures, usually around 130 to 140 F. When compared to a more traditional boiler efficiency of 88% at 180 F, the gas savings can be phenomenal. This may be the only consideration in determining a reasonable return. While true if the evaluation assumes a static point and only boiler efficiencies are evaluated, there are other costs to consider.
Air handling unit (AHU) coils will require more rows for heat transfer. This creates a higher air pressure drop that will require more fan horsepower. There are added costs for larger piping and larger pumps because more water is needed to achieve the same heat transfer characteristics of a higher temperature system. This is especially true in colder climates. Added costs also may include coil freeze protection. The overall first cost of the total system may overshadow the energy savings. Another point to consider is the seasonal operating curve when evaluating equipment; for the analysis to have any validity, part load efficiencies and hours operated at those part loads must be considered.
As an example, assume a building has a 4,000,000 Btu heating requirement. At design conditions a 95% boiler with 130 F supply temperature and a 20 F temperature drop will consume 3.35 therms of gas per hour less than will a boiler operating at 88% efficiency with a 180 F supply temperature and a 40 F temperature drop. This would equate to $3.85 savings per hour at $1.15 per therm of gas. The high-efficiency boiler system would be required to pump 400 gpm using a 5-in. pipe versus the 88% boiler system flow of 200 gpm using a 4-in. pipe. This required additional costs for pipe and pumps; assuming a constant head of 80 ft WC, the difference in pump sizes is 5 hp. The additional operating cost at $0.15 per hour is $0.56. The 130 F supply water system will require a six-row heating coil at 185,000 cfm with an air pressure drop of 4.0 in. WC. The 180 F water system would require a four-row coil with a pressure drop of 3.5 in. This results in a 20 hp difference for the fan motor.
Table 1: Sample cost comparisons
The net operating hourly cost difference at design conditions is $1.06 per hour at $0.15 kWh. The added cost of the pipe, coils, and fan and pump motors, plus the premium for the high-efficiency boiler is $181,900. If a weighted average of 2500 hours per year is assumed, the annual savings is $2,650. This results in a simple payback of 68 years. Although this method is not the most in-depth analysis—a computer program could evaluate part-load performance throughout a year’s operation—it is a reasonable snapshot that should help determine if the system should be considered. Other applications, such as radiant heating, may prove more favorable results based on the inherent design considerations.
The final factors
Another evaluation factor has intangible attributes: the perception, or “I want it,” factor. This does not have a numerical value or formula but must somehow be taken into consideration when evaluating an investment.
If the world of consultants were polled today, it is reasonable to expect that many of us have introduced additional systems that incorporate equipment or design features to get that last LEED point at the owner’s request, and not because it made financial sense. LEED certification can increase an asset’s value and attract tenants, so extra LEED points may make financial sense outside of an engineering perspective.
With insight into total system operation and a general sense of what is valuable added value, a reasonable and simple method can be used to determine the rate of return. There will, of course, be times when more complex methods are appropriate by keeping things realistic. Unfortunately, no one can predict with accuracy what will happen in five years,
Peter D. Zak is a principal with Graef-USA Inc., where he manages the MEP group. He is a member of NCEES and is on the editorial advisory board of Consulting-Specifying Engineer. He was an adjunct assistant professor at the Milwaukee School of Engineering for 20 years and is a registered professional engineer in 24 states.