Could a Fukushima-type disaster happen here?
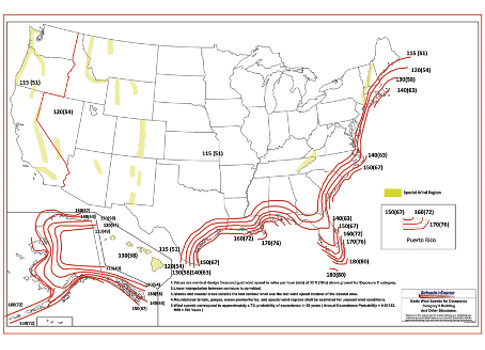
In March 2011, a 9.0 earthquake followed by a tsunami shocked Japan’s northern shore. The devastation to four nuclear reactors in Fukushima caused a state of emergency. The on-site power generators at the plant survived the shake only to be disabled by the tsunami. With no backup power, the cooling pumps could not operate, and without power, the ability to cool the core was disabled, which led to core meltdown. In 2012, Hurricane Sandy hit the northeastern seaboard, crippling emergency power as wind and flooding knocked out many on-site power systems for critical facilities. How prepared are we to maintain emergency power in the wake of natural disasters, such as earthquakes, wind, and floods? Can we limit our vulnerability?
Extreme, but not uncommon
It can be easy to be lulled into a false sense of comfort that "extreme" equals "uncommon." But natural events are fairly common with a number of devastating natural disasters occurring in recent years.
Earthquakes: Worldwide, there is an average of 100 earthquakes per year resulting in damage. In 2013, there were two 7.0 to 7.9 earthquakes in the U.S. and three 6.0 to 6.9. In 2012, there were five 6.0 to 6.9 and 27 that were 5.0 to 5.9. West Virginia experienced a 5.8 in August 2011 that was felt in a dozen states, damaging the Washington Monument and the National Cathedral in Washington D.C., and triggering a shutdown of two reactors at the North Anna nuclear power plant near Mineral, Va. Fortunately, the plant’s four emergency diesel generators started and supplied power to important electrical equipment while off-site power was down. On Jan. 12, 2010, a 7.0 earthquake hit Haiti, leveling much of Port-au-Prince. On Feb. 27, 2010, an 8.8 in Chile toppled entire buildings. The city of Christchurch, New Zealand, was rocked by a 7.0 in late 2010 followed by a targeted 6.3 in February 2011 that destroyed hundreds of buildings. The 1994 Northridge earthquake near Los Angeles, Calif., caused nearly $80 billion in damage. Power outages were experienced as far as British Columbia, Montana, Idaho, Oregon, Washington, and Wyoming-in some cases for up to three days, and intermittently up to a week (see "Earthquake states, statistics").
Wind: High winds from hurricanes, tornadoes, nor’easters, and severe storms affect emergency power every year. Approximately 10,000 severe thunderstorms and more than 1,000 tornadoes occur each year in the U.S.-more than any other country (see Figure 1). There have been an average of 33 killer tornadoes per year between 2011 and 2014. The busiest calendar day for tornadoes was April 27, 2011 (see Figure 2). Millions of customers had power knocked out, including three county emergency operation centers and a 911 dispatch center in Alabama. Some areas took more than a week to restore power. Joplin, Mo., was devastated by the worst tornado since 1947 with more than 150 fatalities and $2.8 billion in damage. In 2004, there were 1,817 tornadoes-a new record. An average of 17 hurricanes per decade have hit the U.S. In 2013, there were 13 named tropical storms in the Atlantic and 18 in the Pacific-11 of these storms developed into hurricanes. Hurricanes Sandy in 2012, Ike in 2008, and Katrina in 2005 were the costliest in damage to date in the U.S. In 2005, there were 27 named storms, 15 of which became hurricanes (see Table 1).
Floods: Floods are the most common disaster in the U.S. More than 70% of presidential disaster declarations are for flooding. Floods are caused by riverine flooding, resulting from overflow of rivers, waterways, dams, etc. when rainfall or snowmelt exceeds their capacity. They are also caused by coastal flooding due to storm surges caused by hurricanes, tropical storms, nor’easters, and tsunamis. In 2011, the Missouri River experienced widespread flooding due to snow melt and rain. Fort Calhoun nuclear power station near Blair, Neb., had to install temporary levees to protect financial assets. Portable fuel trucks were installed at a height above the flood plane to ensure availability of fuel if there was a loss of offsite power. Flood surges in Hurricanes Sandy, Ike, and Katrina severely affected emergency power. During 2008, record precipitation, coupled with already saturated soils, resulted in flooding along many rivers in the U.S. Midwest. Flooding events occurred from January to September of 2008. Substantial record flooding and damage occurred in Illinois, Indiana, Iowa, Kansas, Michigan, Minnesota, Missouri, Nebraska, Oklahoma, South Dakota, and Wisconsin. During 2008, peak-of-record stream flows were recorded at more than 147 U.S. Geological Survey (USGS) stream gages. Emergency generators at several law enforcement agencies were destroyed by floodwaters. Figure 3 shows a USGS map of stream gage measurements across the U.S. from 2008 to 2013.
There is no shortage of natural disasters. All 50 states experience some sort of natural disaster that knocks out daily utility power. Facilities, especially critical facilities that are essential to provide necessary services after a disaster, must keep on-site backup power available to continue operation in the absence of utility power. It is crucial to design and install on-site emergency power systems to survive these disasters.
Emergency power vulnerabilities
Earthquakes, wind, and floods are the forces of nature responsible for challenging the vulnerabilities of emergency and backup power systems. However, taking the appropriate precautions can help guard against these vulnerabilities.
Earthquakes: During an earthquake, the ground motion creates a dynamic response in a building and its equipment. This interaction creates an acceleration applied to equipment that results in a sometimes violent shaking. This effect can increase up to a factor of three for equipment mounted on the roof. Emergency generator systems must be capable of withstanding imposed seismic loads. They must be sufficiently anchored to resist seismic loads with no parts becoming detached from the building. They must also accommodate differential movement between the equipment and connected systems, such as exhaust systems, fuel lines, wiring, and process pipes such as coolant.
Emergency generators that are properly anchored typically perform well. However, common failures experienced in recent earthquakes include:
- Off-site natural gas fuel supply was interrupted
- Generator failed to operate due to component damage
- Anchors missing
- Battery backups not anchored
- Fuel tank not anchored
- Exhaust stack not braced
- Fuel oil pipe not braced
- Failure of isolator supports that were not seismically rated or anchored
- Lack of flex connectors between the unit and distribution systems.
For emergency power to function, interconnected systems require attention too. This includes ancillary equipment such as starters, fuel and lubrication pumps, cooling equipment, and more. Distribution systems, such as switchgear, panels, controllers, feeders, branch circuits, transfer switches, transformers, and so on, need to function. Failure of any component in the emergency power system can prevent its operation. All components of the system must be braced for seismic loads.
Wind: Damage to on-site power generating equipment by wind forces has increased concern about the adequacy of equipment protection. Wind effects must be evaluated for two main areas of the power generating equipment: the equipment that is exposed to wind and the exterior wall-mounted cladding components, such as intake and exhaust louvers. Protection from wind-borne missiles (debris) is a design consideration but not a code issue. The barriers that protect the power generating equipment can be designed for missiles. Louvers that need to operate are especially vulnerable. Exhaust stacks and any part of the equipment that may be unprotected should be part of the design considerations. Generally, on-site power equipment resists wind well if anchored properly. However, some vulnerabilities to wind include:
- No on-site emergency power; if no emergency power is in place, critical facilities can offer only limited or no service
- Not anchored properly
- Fuel tank not anchored properly
- Remote radiator not anchored properly
- Enclosure or louvers not capable of wind loads
- Exhaust muffler not anchored properly for wind load.
Floods: Flood damage poses one of the greatest risks to on-site power. The most common reason for flood-damaged emergency generators is location. Mechanical and electrical equipment is often located in lower levels of a building. The lowest level of the building is also the most likely to flood. Floodwater can damage or inundate fuel tanks that supply diesel generators, damage fuel oil pumping equipment, and knock out emergency power distribution equipment, such as transfer switches, panels, and feeders. After Hurricane Katrina, generators in a hospital were elevated but the transfer switches were not. The switches were rendered inoperable by flooding. Although the generators could function, there was no way to transfer the power to critical equipment. Many of a major medical campus’s emergency generators were located in lower levels and flooded during Hurricane Ike. These buildings could not provide many critical services. In one building, the generator and associated equipment were mounted on the second floor. High and dry, they remained operable during and after the flood.
Similar issues rendered emergency power in critical facilities useless during the 2008 Midwest floods. In one instance, bucket brigades formed a line up 14 flights of stairs to carry diesel fuel to the generators. The fuel pumps had been located on a lower level and were destroyed by floodwaters. Fuel supply is an important design consideration for emergency power equipment. Both above ground and buried tanks have additional flooding concerns that need to be addressed. Buried tanks need to be evaluated for the additional hydrostatic pressure of the water levels above grade. Aboveground tanks must be anchored and elevated so they are not swept away by moving water. The anchors also restrain the tank as the fuel is used where the void will cause a buoyancy uplift. Both aboveground and buried tanks need to be evaluated for the flood level and operation. The weight of the water could cause a failure of a buried tank from the hydrostatic load. If the floodwater is going to stay for an extended period of time, refueling is an issue. All tanks are vented, so the vent pipe must be extended above the potential flood levels.
Building code
Since the introduction of the International Building Code (IBC) in 2000, it has been updated based on lessons learned in natural disasters. It is updated every 3 years. All 50 states have adopted it and most are currently using the 2009 or 2012 version. The good news is that following the IBC works. An investigation of the U.S. Embassy in Port-au-Prince after the 2010 Haiti earthquake found little damage and the building was fully operational. The building specification required strict adherence to the IBC. As a result, while the 7.0 earthquake demolished most buildings, the building and equipment built to the IBC performed well. A study of 9,835 reported claims in Hurricane Rita conducted by the Texas Windstorm Insurance Association shows that building codes work. There were far fewer claims reported for dwellings built to the new codes, and the average paid loss per dwelling was 40% to 50% less.
The seismic and wind load design provisions in the IBC are based on the American Society of Civil Engineers (ASCE) 7: Minimum Design Loads for Buildings and Other Structures. The 2006 and 2009 editions of the IBC refer specifically to the 2005 edition of ASCE 7 (i.e., ASCE 7-05), and the 2012 edition of the IBC refers to the 2010 edition of ASCE 7 (i.e., ASCE 7-10).
There are two code criteria that dictate when emergency generators must comply with IBC requirements for seismic design: the seismic design category (SDC) and the equipment importance factor (Ip).
SDC: SDC is a classification assigned to a structure based on its occupancy or risk category (emergency facility, public assembly, warehouse, etc.), the severity of the earthquake ground motion at the site as defined by the IBC map (similar to Figure 4), and the soil stiffness (rock solid or shifting sand). The SDC ranges from A to F. A is the least severe; F is the most severe. We won’t go into all the details of how the SDC is determined, as that could be an article unto itself. The registered design professional for the project provides the SDC classification. The IBC requires that the general information page of the structural drawings list the SDC. It is typically on the first page of the structural drawings.
Equipment Ip: Each piece of equipment is given an equipment Ip that indicates if it is important for the equipment to be operating after an earthquake. If the equipment is important or hazardous, then it is designated as Ip = 1.5. If it is not important or hazardous, then it is designated as Ip = 1.0. All equipment is assigned an importance factor based on the following:
The Ip shall be 1.5 if any of the following conditions apply:
- The component is required to function for life safety purposes after an earthquake, including fire-protection sprinkler systems and egress stairways.
- The component conveys, supports, or otherwise contains toxic, highly toxic, or explosive substances, where the quantity of the material exceeds a threshold quantity established by the authority having jurisdiction (AHJ) and is sufficient to pose a threat to the public.
- The component is in or attached to a Risk Category IV structure (emergency facility) and it is needed for continued operation of the facility.
- The component conveys, supports, or otherwise contains hazardous substances and is attached to a structure or portion thereof classified by the AHJ as a hazardous occupancy.
All other components shall be assigned a component Ip equal to 1.0.
While the IBC gives direction on minimum Ip requirements, the design professional in conjunction with the building owner assigns an Ip based on the anticipated use of the equipment and its need to operate post-earthquake. An Ip of 1.5 may be based on risk of the equipment’s economic loss and its vital role in business continuity if the equipment fails.Typical Risk Category IV buildings where the generator Ip would be 1.5 include:
- Hospitals and emergency treatment facilities
- Police, fire, ambulance, emergency response (911), and recue buildings
- Water facilities required for fire suppression
- Public power generating stations and utilities
- Aviation control towers
- Facilities critical to national defense
- Designated public storm shelters
- Data centers
- Facilities with toxic or hazardous substances.
Use the SDC and the Ip in conjunction with Table 2 to determine if seismic compliance is required. If the equipment is exempt, there are no special seismic requirements. If the equipment is not exempt and has an importance factor of 1.0 (nonessential), the equipment shall be properly anchored for seismic loads. If Ip = 1.5, the IBC requires emergency generators to be proven to be functional after an earthquake by testing or analysis and requires a label by an approved agency. Active equipment with dynamic moving or rotating parts, or parts that are energized (generators, motors, pumps, switchgear, etc.) must be tested using a shake table per ASCE 7, Chapter 13, Section 2.2. Nonactive parts (enclosures, stands, tanks, etc.) may be analyzed for seismic compliance per ASCE 7, Section 13.2.1, and force requirements per ASCE 7, Section 13.3. Interaction between the equipment, connected pipe or conduit, the supporting structures, and other mechanical and electrical equipment shall also be included.
Emergency generators must also be evaluated for wind forces in accordance with the IBC. The design wind force is an equivalent static force that is assumed to act on a component in a direction parallel to the wind and not necessarily normal to the surface area of the component. This force varies with respect to wind velocity and to heights above ground level. On some projects, wind loads are greater than earthquake loads and therefore they govern. These loads are directly related to the surface area, shape, and size. There are no exemptions for wind. All enclosures, claddings, and exhaust components must be designed to withstand the velocity pressure applied per the IBC. Velocities range from 85 to 200 mph, depending on the project location and importance (see Figure 5). Pressures range from a minimum of 10 lb/sq ft to more than 175 lb/sq ft depending on the required wind velocity, exposure, height above grade, terrain, and wind importance factor (IW). IW is independent of the seismic Ip (see Table 3). Table 4 provides a sampling of wind pressures calculated per equations in ASCE 7-10. It is important to note that wind loads for IBC 2000 to 2009 are per ASCE 7-05. In IBC 2012, wind velocities are calculated per ASCE 7-10. In ASCE 7-10 wind velocities increased, the chapter changed from 6 to 23, and the method of calculation changed. So it is important to use the governing/accepted version of the IBC. Local jurisdictions will sometimes increase the wind velocity based on special wind regions or added safety factors.
Design and construction in flood hazard areas is regulated by Chapter 5 of ASCE-7 and ASCE 24-05: Flood Resistant Design and Construction. ASCE-24-05 requires utilities to be either elevated or dry flood proofed. It is recommended to move the equipment high in the building out of the flood levels. If this is not possible, building wall construction can be provided to slow the floodwaters, but supplemental pumping may be required. Base flood elevation is the elevation of flooding, including wave height, having a 1% chance of being equaled or exceeded in any given year. Flood insurance rate map (FIRM) is an official map of a community on which the Federal Insurance and Mitigation Administration has delineated both special flood hazard areas and the risk premium zones applicable to the community. It designates coastal zones and coastal high hazard areas. The design flood and design flood elevation is the greater of the following:
- The base flood, affecting those areas identified as special flood hazard areas on the community’s FIRM
- The flood corresponding to the local jurisdiction legally designated flood hazard area on a community’s flood hazard map.
Hydrostatic pressures and wave loads are as required in chapter 5 of ASCE 7.
Certification
Chapter 17 of the IBC requires designated equipment (essential to operate) to be certified for seismic force requirements. Manufacturers must test or analyze the component and its mounting system or anchorage and submit a certificate of compliance for approval by the building official. Qualification of active mechanical and electrical equipment shall be certified by approved shake table testing. Static items, such as tanks or enclosures, may be qualified by an analytical method using dynamic characteristics. Shake table testing must be per a recognized test method such as ICC-ES AC 156, which provides detailed procedures for seismic qualification by testing. EGSA Recommended Practice for Seismic and Wind Certification provides helpful direction for certifying emergency generators.
The code requires certification by an established and recognized agency regularly engaged in conducting tests, analysis, qualification, and inspection services for seismic certification. To ensure credibility, follow ISO standards for certification or listing agencies that require written assurance (certificate) by an independent, accredited external body that audits the manufacturer’s management system, verifies conformance to a standard, and records certification in a public register. An accredited inspection body is formally recognized and audited by an accreditation body (IAS, International Accreditation Service) to ensure a certification or listing agency is competent to perform the work per ISO 17020.
There is a difference between qualification and certification. Qualification determines if a sample product supplied by a manufacturer meets seismic and/or wind load requirements by test or analysis. This is just the first step
An average of 17 earthquakes ranging from 7.0 to 7.9 and one at greater than 8.0 occur each year. Every state in the U.S. has earthquakes.toward certification. Certification is a combination of testing and quality assurance inspection. For certification, an accredited third-party reviews the qualification for compliance to industry standards, and inspects the manufacturer’s quality control program to ensure future products will meet the original qualification or product performance. To maintain a certification/listing, an accredited agency must regularly inspect the manufacturing facility (annually or as needed). A true certification process encourages manufacturers to play by the same rules, providing risk protection to registered design professionals and peace of mind to building owners and AHJs.
Chapter 17 of the IBC requires the manufacturer to apply a label on a certified product that includes the name of the manufacturer, functional performance, and name of the agency responsible for ensuring that a representative sample of product has been tested and/or evaluated by the approved agency.
Weathering the storm Natural disasters are inevitable, and power is likely to be lost in areas hit by disasters. It is important for critical facilities to have standby emergency power to maintain essential services afterward. Fortunately, we can be prepared to weather such extreme events. If we adhere to building code requirements, follow good installation practices, specify certified products, and inspect the installation, we can rest assured that the emergency power will be available to support critical services after the disaster.
Earthquake states, statistics
- There are 39 states at risk of moderate to major earthquakes
- The U.S. has 10 moderate earthquakes each year.
- There is a 40% to 60% chance of a magnitude 6 earthquake within 15 yr in the New Madrid region, which stretches from Memphis, Tenn. to St. Louis.
- A magnitude 5.5 to 6.5 occurs in Utah every 7 to 10 yr.
Large earthquakes in U.S. history
- New Madrid, Mo.: Four earthquakes occurred between December 1811 and February 1812 ranging from 7.0 to 8.1. They were felt over the entire eastern U.S. (more than 2 million sq mi), with Missouri, Tennessee, Kentucky, Indiana, Illinois, Ohio, Alabama, Arkansas, and Mississippi experiencing the strongest ground shaking.
- Charleston, S.C.: August 1886, magnitude 7.7
- San Francisco: April 1906, magnitude 8.3
- Olympia, Wash.: April 1949, magnitude 7.1
- Hebgen Lake, Mont.: August 1959, magnitude 7.3, also known as the 1959 Yellowstone earthquake, which created Quake Lake
- Prince William Sound, Alaska: March 1964, magnitude 8.4
- Borah Peak, Idaho: October 1983, magnitude 7.0
- Santa Cruz, Calif. (Loma Preita): October, 1989, magnitude 7.0, $10 billion in damages.
Robert E. Simmons, PE, is CEO of Seismic Source International, LLC. He has more than 25 years of experience in design, development, and application of vibration and shock isolation, and seismic/wind restraint of nonstructural components, such as mechanical, electrical, plumbing, and fire protection equipment and related systems.
James A Carlson, PE is president of Seismic Source International, LLC. He has more than 30 years of experience in industrial and nuclear facilities designing resolutions to technical problems for the nuclear industry, seismic/wind restraint design for nonstructural components, seismic qualification of equipment, and quality inspection services.