Performance-based design allows design teams creative freedom when integrating energy efficiency strategies into a facility.
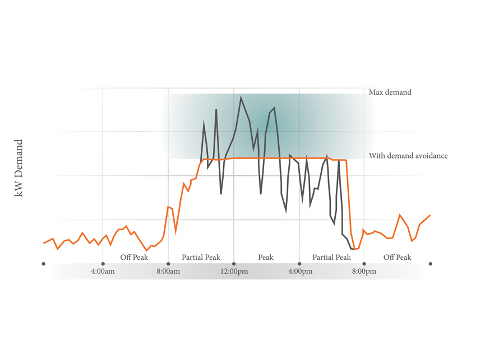
Learning objectives:
- Illustrate the methods to evaluate building energy consumption.
- Apply performance-based design methods to limit energy consumption via products or systems.
- Learn about performance criteria that address building efficiency: energy utilization index and electric demand ratio.
Performance-based design criteria are gaining popularity in the United States as macroscopic means of improving building efficiency. This type of approach is used by some owners and supported by sustainable design guidelines and energy codes, to allow design teams creative freedom integrating energy efficiency strategies into a facility. The energy-control measures commonly proposed by these performance-based requirements focus on reducing overall energy consumption or limiting the peak electric demand. Architecture 2030 is a popular example of a holistic performance-based design criterion for the reduction of carbon emissions from the built environment.
Building energy consumption can be evaluated using an energy utilization index (EUI). The EUI is usually expressed in total energy consumed in a year divided by the floor area of the building. The sources in the EUI calculation include electricity, natural gas, fuel oil, and any other fuel used by the building. Energy created by renewable sources, such as photovoltaic energy, is usually not included in the EUI calculation. A common unit of measure for EUI is 1,000 x Btu (kBtu) per square foot per year. There are many resources, among them Commercial Buildings Energy Consumption Survey (CBECS), that catalog average EUI values for building types and locations.
An example of how the performance-based criteria can be applied is a stated requirement that limits the building consumption to 30% better than the 2012 CBECS average. In response, the design team uses this requirement when specifying energy-consuming components and systems related to the building, such as the envelope, HVAC, and lighting systems. An aggressive EUI performance requirement demands a holistic approach to design. The careful selection of building components that take advantage of the natural features available can reduce the need for energy-consuming systems.
An alternative approach to performance-based design can be achieved through limiting the peak electric demand of a facility. The techniques associated with this approach can be (and often are) coupled with the EUI strategy to maximize efficiency of the building.
Utility companies use cost strategies for electric demand to manage peak consumption and the effect that it can have on the electric infrastructure. These rate structures vary by region and utility company, but the premise is the same. For commercial customers, utility companies include the demand charge for maximum power used during a period, measured in dollars per kilowatt, in addition to the charge for electricity consumed, measured in dollars per kilowatt-hour. Design strategies that take advantage of electric demand rate structures to reduce operating costs are not new.
These designs have been most commonly applied in facilities that are very large power users, like hospitals and other mission critical facilities. Building types such as education facilities can experience costs associated with demand changes as high as 50% of the overall electric bills. The peak electric demand in education facilities is short-lived—30 minutes or less—and a result of building systems and food service simultaneously operating at the maximum level. As a result, a wider range of owners and developers are including peak electric demand performance criteria in their building designs.
Electric demand control
The electric demand control limits are measured through an electric demand ratio (EDR) for a building. EDR is usually expressed in maximum electricity consumed in a defined interval for a billing cycle divided by the floor area of the building. The defined interval and billing cycle are normally consistent with the electric rate structure applied to the facility. A common unit of measure for EDR is watt per square foot. Demand rate structures vary widely depending on many factors, such as service provider, time of day, and month of year. The important criteria for the design team is to select systems, equipment, and control strategies that take advantage of these rate structures.
An example of how this approach can be applied is when an owner defines a maximum EDR for a building. In response, the design team uses this requirement when specifying building energy-consuming components and systems, such as HVAC, lighting, and equipment control systems. There are opportunities for architectural features of the building to affect this approach, but a bulk of the responsibility for success is associated with the engineered systems.
The approach to performance-based design strategies must include a verification stage where the actual energy consumption is compared with the prescribed guidelines once the project is complete and a history of quantifiable data is available. The commissioning of a building will play an important role in the success of achieving the performance-based design criteria. The design team often is required to evaluate these comparisons and make recommendations to resolve any inconsistencies that exist.
Electric demand control can be used to satisfy performance-based design criteria for new construction, renovation projects, and standalone applications. This approach is most popular in renovation or standalone projects, because the application tends to be engineered system-related and have attractive paybacks. The outcome for this type of project also is quantifiable, because the history of energy consumption and the utility rate structures are known. This approach focuses on electricity use, making it widely applicable to lighting, cooling systems, and buildings with electric heat. An approach to limiting electric demand can be accomplished using sophisticated control strategies or energy-storage equipment that manipulate system operation to avoid the peaks. A facility must be outfitted with a building automation system (BAS) that monitors an electric-power meter to automatically manage the operation.
Energy benchmarks
The project example used throughout this article is a 327,000-sq-ft high school located in a rural community west of the Kansas City metro area (see Figure 1). This building is located in ASHRAE Climate Zone 4.
The first step in creating performance-based design criteria limiting peak electrical consumption is to perform an energy benchmark using electric bills. This benchmark is used to understand how the rate structure is applied and the level of existing consumption. The peak electric values that trigger demand cost are of interest. From past analysis, it is known that reducing the peaks will also reduce overall electric consumption.
Table 1 shows an electric demand profile for the example K-12 education facility. This building uses gas-fired boilers for heat, so most of the electricity is used for lighting, cooling, and plug loads. The values provided in Table 1 for EDR and corresponding demand-cost ratio have an order of magnitude and pattern typical for this facility type in this climate.
Following benchmarking, the next step is to use data from the building power meter to understand the consumption profile and specific peak characteristics. Figure 2 shows an electric-consumption profile for a typical K-12 educational facility. This is useful for setting the limits for reduction of peak consumption.
The source of the peak electric demand depends on the building personality, but there is evidence that peaks are not set by individual systems. Using the K-12 example, peak electric demand is rarely discovered when the building becomes occupied in the morning or during the heat of the afternoon. These times may require a big effort from the HVAC equipment, but the HVAC equipment alone does not set the consumption peak. It is more common for the peak electric demand to be set when all systems are fully operational and the occupants are present and engaged in activities. In K-12 facilities, peak electric demand typically occurs between 11 a.m. and 1 p.m. An office, hospital, or other building type may have a different energy personality.
There is some finesse required when determining the performance-based limits for peak electric demand consumption. The approach is represented in Figure 3 by drawing a line at the maximum allowable consumption point. If the line is drawn too high on the graph, then the opportunity to maximize cost avoidance is lost. An aggressively applied limit will result in decreased occupant comfort or other operational issues. The approach for any demand-control strategy should be so subtle that building occupants don’t detect the adjustments. The following guidelines should be used for applying electric demand limits:
- Allow limits to be adjustable for future fine-tuning.
- Vary the limits to take advantage of rate structures and building personality.
- Apply the demand limits incrementally.
- Use notification alerts to denote when limits are being applied.
- Train building operators and educate occupants.
Design strategy: control
The most common approach to electric demand control uses sophisticated strategies. In many cases, the peak electric demand being avoided has a duration of less than 30 minutes, occurs once or twice a day, and represents about a 20% increase above the value measured on either side of the apex. The design strategies used to achieve performance goals are site-specific due to the unique combination of engineered systems present, climate factors, and other facility features. The systems affected by this approach include lighting and HVAC, both hydronic and airside.
A common strategy is to manipulate the temperature setpoints to reduce the load imposed by the HVAC system. This application uses the BAS to automatically adjust temperature setpoints as a means of avoiding peak electric demand. The unique part of the design is determining which setpoints to adjust so the outcome is undetectable by the occupants while performance goals are achieved. Airside systems can be hardest to manage because of the direct impact on the occupied space. There must be a balance of selecting systems or zones to achieve the electric demand goals and indoor environmental management.
It is recommended that this strategy be staged for gradual implementation and start with equipment serving larger zones. Larger zones offer the best opportunity for consumption avoidance because larger environments react slower to change, thus is less detectable by occupants. The other important part of this strategy is to recover from the setback carefully to avoid creating artificial peaks caused by maximum equipment operation.
Table 2 provides a summary for how this approach can be applied in the example facility. The rate structure for electric demand is applied monthly at $10/kW from June through September and $8/kW for the remaining months of the year. This utility company measures the electric demand in 15-minute intervals. Timing control sequences to correspond with measurement timing is hardly possible and not recommended. However, both the rate structure and frequency are important for determining a strategy.
A computer simulation was used to determine that approximately $24,000 savings per year could be generated through managing the peak electric demand. A simple return on investment (ROI) on a 2-year payback cycle would allow for $48,000 as a budget for implementing design strategies. A reasonable amount, considering the scope of work would include a power meter and relatively simple programming to the BAS.
Design strategy: equipment
Energy-storage systems are another way to achieve performance-based design criteria through electric demand control. A true demand-control application always uses a control sequence component. The inclusion of storage equipment can offer additional benefits when higher levels of performance are desired.
Thermal-storage systems have been used for decades as a strategy for managing peak electric consumption and providing a level of redundancy. The broadest application of this technology comes in the form of customized cold water or ice storage that complements the operation of a chilled-water system. In the past, the cost and complication of these systems have limited the use to large mission critical applications or higher education campuses, where investment and staff are available to successfully operate these systems. The emergence of modular, more affordable products with manageable maintenance routines has opened the application of ice-storage systems to a wider range of building owners.
Figure 4 provides a simple flow schematic for how an ice-storage system can be applied. The design strategy is to charge the ice-storage tanks with chilled-water equipment when the building use is low—for most facilities, this is in the evening. When the ice system is being recharged, the other electrical systems associated with the facility are lightly used so operating the chilled-water equipment should not jeopardize the peak-reduction strategy. The ice-storage equipment is allowed to discharge, rather than operating the conventional chilled-water equipment to manage the expected peak electric consumption. This cycle repeats daily during the cooling season.
For simplicity, this example is shown with air-cooled chilled-water equipment with omitted redundant equipment and instrumentation. A primary-secondary pumping arrangement is used. The following sequence is applicable for chilled-water equipment operation:
Normal chilled-water system operation:
- V1B open, V1A closed
- PP-1 enabled
- Chiller enabled
- V2B open, V2A closed
- PP-2 disabled
- V3B open, V3A closed
- SP-1 enabled, variable with load.
Chilled-water equipment charges the ice-storage system:
- V1B open, V1A closed
- PP-1 enabled
- Chiller enabled
- V2B open, V2A open
- PP-2 enabled
- V3B closed, V3A open
- SP-1 normally disabled.
Ice-storage system provides cooling:
- V1B closed, V1A open
- PP-1 disabled
- Chiller disabled
- V2B closed, V2A open
- PP-2 enabled
- V3B closed, V3A open
- SP-1 enabled, variable with load.
There will be periods at the end of the ice-storage discharge when the chilled-water temperature is not satisfactory to serve the cooling load. It is possible to operate the system with the chilled-water and ice-storage systems enabled simultaneously.
Ice-storage system and chillers provide cooling:
- V1B open, V1A closed
- PP-1 enabled
- Chiller enabled
- V2B closed, V2A open
- PP-2 enabled
- V3B and V3A modulated in opposite position
- SP-1 enabled, variable with load.
To maximize the benefit of the cooling equipment, the system design and control sequence must include the combined operation of the ice-storage and chilled-water systems. Systems are normally tested and setup to operate independently—chillers only or ice storage only—in steady-state condition. The challenge for a successful project is seamless transition in operating modes without losing control of the facility. Engineers will need to consider speed control for pumps, modulating valves, and transient equipment parameters in the system design.
Reducing cost, energy consumption
The approach for selecting the size of the ice-storage system can go a long way to determining its viability. Similar to the approach described for the control system modifications, an ice-storage system with too much capacity will have no trouble fulfilling the performance goals, but will likely exceed any budget limitations that exist. A system selection that is too small may be cost-effective, but will not be robust enough to manage the peak electric demand to the desired level.
The cost of these systems suggests that the goal for electric-demand avoidance is greater than shaving the apex of the consumption graph. The selection strategy for the ice-storage system must include a significant reduction of the electric consumption and demand to create enough cost avoidance to make the ROI attractive.
Table 3 provides a summary of the analysis for the project example. A computer simulation of the facility and the cooling system was developed to test multiple parameters of the ice-storage system. The capacity of the ice storage system was varied to determine how the overall energy consumption and electric demand was affected. The computer simulation was calibrated to represent actual electric utility data gathered during the benchmarking process.
The owner’s goal in employing this system was to reduce the energy consumption and manage the demand charges for the facility. The approach was to vary the amount of ice storage in the computer simulation until there was no more impact on the electric demand. At this point, it was expected that the electric demand created by the chillers was eliminated. This point is referred to as “full ice.” “Half ice” was arbitrarily selected as an intermediate condition with half of the capacity as the “full ice” condition. The base case for this evaluation assumed no ice-storage equipment.
For this example, a simple payback of approximately 10 years was calculated. The rate structure for this example was fairly modest as compared with other parts of the United States. Electric demand was applied monthly at a rate of $10/kW from June through September and $8/kW for the remaining months of the year. The cost of energy was $0.072/kWh. This ROI may not be attractive for some building owners. Typically, building owners that fund operational costs separately from capital costs and plan to own the facility indefinitely have an appetite for longer payback periods.
Long-term energy goals
Building owners, sustainable guidelines, and energy codes are turning to performance-based design criteria to promote energy reduction and creativity by design professionals. Two of the most popular performance criteria that address building efficiency are EUI and EDR limitations.
EUI is a commonly used ratio that normalizes total energy consumed by the building. Design professionals typically use a holistic approach that incorporates architecture and engineered systems to develop high-performing facilities with aggressive EUI targets. EUI reductions impact the amount of energy used and the cost avoidance of using less energy. Performance-based design criteria that use EUI targets are normally applied to new construction or major renovations projects.
EDR is another normalized ratio that quantifies the electricity consumed at a moment in time. The primary focus of an EDR limit is to avoid utility-imposed demand charges. The approach is typically limited to electrical systems since demand charges are usually imposed by electric utilities. The reduction in overall energy consumption is a secondary outcome. Demand-control reduction strategies can be easily applied to new, major renovation, or standalone projects.
Many performance-based design criteria rely on sophisticated equipment and sequence of operation. The attempt of promoting energy efficiency and cost avoidance can be derailed if the systems are not started up and operated properly. An important part of achieving successful ownership falls on the commissioning team, properly trained building operators, and educated occupants. The performance-based design criteria examples discussed in this article rely on actual operating data to verify compliance with the requirements. A commissioning plan that includes some type of ongoing review of operation and performance is necessary to ensure the investment is realized for the life of the building.
Many of the equipment, systems, and sequences employed by these strategies rely on occupants to understand the impact of their actions. Occupant behavior is another component of ensuring the full potential of a high-performing facility. The energy performance of a building is highly dependent on the choices the occupant makes regarding the proper use and maintenance of building systems. Traditionally, the design team’s role on a project ends when the building is built and all of the post-construction documentation is turned over to the owner.
Systems in modern-day buildings are complicated, especially for users with little to no training in architecture or engineering. Systems that are not understood are often incorrectly altered by occupants and ultimately negatively affect energy consumption. Design, commissioning, and energy professionals can positively impact building performance by educating occupants on how their systems work.
Rodney V. Oathout is an energy and engineering leader and principal at DLR Group. He is a champion of integrated design, energy efficiency, and human engagement in high-performance building design. He is a member of the Consulting-Specifying Engineer editorial advisory board.